Boosting the sensitivity of a red-light-responsive histidine kinase
We recently engineered the chimeric SHK DrF1 as a fusion between the photosensory module (PSM) of the D. radiodurans BphP and the DHp/CA effector moiety of Bradyrhizobium japonicum FixL (Fig. 1b)4,28. Combined with the cognate RR FixJ from B. japonicum, DrF1 forms a TCS and affords gene expression from target promoters that can be dialed down by over 200-fold under red light compared to darkness. For applications in optogenetics29 and biotechnology, all system components were assembled on the portable plasmid pREDusk28 (Fig. 1b). Using this plasmid as a platform, we explored how replacement of the D. radiodurans PSM (DrPSM) for another PSM impacts on TCS output and response to light. We opted for the PSM of a BphP from Deinococcus maricopensis (DmPSM) because this module recently proved efficient at regulating an adenylyl cyclase, outperforming around twenty other PSMs, including the DrPSM30. Informed by a sequence alignment (Suppl. Fig. 2), we generated the DmF1 SHK by introducing the DmPSM (residues 1-510) in lieu of the DrPSM while keeping the BjFixL fragment (residues 266-505) unaltered (Fig. 1b). Within the pREDusk context, DmF1 drove expression of the red-fluorescent reporter gene DsRed in darkness that was strongly suppressed under red light (650 nm) (Suppl. Fig. 3). In the following, we refer to this setup as DmREDusk, while the original system it derives from28 will be designated as DrREDusk (Suppl. Table 1).
We next assessed bacteria harboring the DmREDusk circuit in depth and found that the reporter fluorescence monotonically decreased with increasing red-light dose by around 300-fold, comparable to DrREDusk (Fig. 1c). With a half-maximal light dose of (0.11 ± 0.01) µW cm−2, DmREDusk intriguingly proved tenfold more light-sensitive than DrREDusk [(1.11 ± 0.02) µW cm−2]. It is worth noting that, with certain exceptions31, the PSMs of BphPs generally exhibit overall quantum yields of around 0.1–0.2 for Pr→Pfr photoconversion32. That is, only 10–20% of the absorbed light quanta trigger a downstream signal response. Although no pertinent experimental data are available for the D. radiodurans BphP, a recent simulation study puts its quantum yield for Pr→Pfr photoconversion at 15%33. Even in the absence of further investigation, it is hence likely that the unexpected and puzzling difference in light sensitivity between DmREDusk and DrREDusk cannot be explained by differing quantum yields for the Pr→Pfr transition, at least not to full extent, as quantum yields generally range between zero and unity.
The striking sensitivity difference also manifested in the pREDawn context that derives from pREDusk and harbors a gene-inversion cassette based on the λ phage cI repressor34 that inverts and amplifies the response to light (Fig. 1b). When exposed to increasing doses of red light, the DsRed reporter expression in bacteria bearing DmREDawn levelled up by around 26-fold at a light intensity of 0.2 µW cm−2, before again dropping threefold at even higher intensities (Fig. 1d). For reference, DrREDawn mediated an increase of DsRed expression by around 61-fold at a light dose of 2.3 µW cm−2, which decreased by twofold at higher light intensities. As reported previously28, the attenuated response at high light intensities likely owes to the presence of the cI repressor and its strong target promoter pR. That notwithstanding, introduction of the DmPSM garnered a 10-fold light sensitivity gain, as it did in the DmREDusk context.
To glean additional insight, we probed the red-light-gated gene-expression circuits by flow cytometry. Under non-inducing conditions, i.e., saturating levels of red light (650 nm, 100 µW cm−2), both DmREDusk and DrREDusk exhibited uniformly low reporter fluorescence that was only 1.6-fold above the background fluorescence of a control circuit bearing a multiple-cloning site (MCS) rather than the fluorescence reporter (Fig. 1e). Transfer to darkness incurred a uniform shift of the single-cell fluorescence distribution to 56-fold higher values. Both the DmREDawn and DrREDawn exhibited some leakiness under non-inducing, dark conditions (Fig. 1f). While the single-cell fluorescence peaked at values around 2.1-fold higher than those of the MCS controls, subpopulations of cells exhibited partial induction with higher reporter fluorescence. We provisionally ascribe these subpopulations to continuous expression of the strong cI repressor which arguably imposes a considerable metabolic burden on the bacteria. Under inducing, red-light conditions, the single-cell fluorescence distributions of DmREDawn and DrREDawn coalesced to uniform populations at around 410-fold and 500-fold higher values, respectively, than the main peak in darkness.
On the whole, the exchange of the DrPSM input module for DmPSM consistently installed higher light sensitivity in both the pREDusk and pREDawn circuits. Higher sensitivity may be advantageous for multiplexing of several optogenetic circuits and for applications where light delivery is limiting.
Photochemical properties alone cannot explain the enhanced light sensitivity
To unravel the underpinnings of the more sensitive light response in the Dm-based vs. the Dr-based gene-expression circuits, we expressed and purified the underlying DmF1 and DrF1 photoreceptors. Both receptors covalently incorporated their biliverdin chromophore to similar extents, as gauged by absorbance spectroscopy (Fig. 2a, b, Suppl. Fig. 4). Upon far-red illumination (800 nm), both DmF1 and DrF1 assumed the Pr state, characterized by the so-called Soret and Q absorbance bands at 400 nm and 700 nm, respectively (Fig. 2a, b). Exposure to red light (650 nm) populated the Pfr state with Soret and Q band maxima at 410 and 752 nm, respectively. As red light also drives the reverse Pfr→Pr transition, if to lesser degree, a photostationary state resulted with Pr:Pfr ratios of 0.17:0.83 for DmF1 and 0.09:0.91 for DrF1. Evidently, red light converted DrF1 to its Pfr state to slightly higher, but overall similar extents compared to DmF1.
We next reasoned that DmF1 and DrF1 may well differ in how efficient red light is at driving their Pr→Pfr transition. At a given light intensity, the velocity of this transition scales with the extinction coefficient at the excitation wavelength times the quantum yield for productive photochemistry leading to biliverdin isomerization11,32. Notably, DmF1 and DrF1 did not substantially differ in the degree of BV incorporation (Suppl. Fig. 4), and they showed similar absorbance properties (see Fig. 2a, b). Hence, for a given intensity of red light, the kinetics of the Pr→Pfr conversion provide an approximate handle on the relative quantum yields in DmF1 and DrF1. We accordingly recorded photoactivation kinetics by illuminating the sample with red light (1 mW cm−2, 650 nm) while monitoring over time the change of the absorbance signal at 750 nm (A750) which reports on the fractional Pfr population. For both DmF1 and DrF1, the monotonic increase of the A750 signal could be described by single-exponential functions with rate constants of 0.036 s−1 and 0.028 s−1, respectively (Fig. 2c). The somewhat faster kinetics in DmF1 hint at a more efficient, light-driven Pr→Pfr transition which, all other things being equal, translates into a higher light sensitivity. However, given its magnitude of around 30%, it appears unlikely that this effect alone can account for the tenfold sensitivity difference evidenced above in the DmREDusk and DrREDusk circuits. We also probed the photoactivation kinetics of the reverse Pfr→Pr process under far-red light (5 mW cm−2, 800 nm). Interestingly and for unknown molecular reasons, these kinetics were around 2.7 times faster in DmF1 relative to DrF1, indicative of a more efficient light-driven return to Pr (Fig. 2c). As the above pREDusk reporter-gene experiments were, however, conducted using only red light, this difference cannot explain the observed sensitivity differences either.
a UV–vis absorbance spectra of DmF1 upon illumination with far-red light (800 nm, purple line) or red light (650 nm, red line). The dotted line denotes the pure Pfr spectrum calculated according to69. All spectra were normalized to the absorbance at 700 nm after far-red light exposure. b As panel a but for DrF1. c The Pr→Pfr photoactivation kinetics of DmF1 (green) and DrF1 (orange) driven by red light (red flash), and the corresponding Pfr→Pr reversion kinetics under far-red light (purple flash) were monitored by the absorbance at 750 nm and evaluated according to single-exponential functions. d The dark recovery of DmF1 (green) and DrF1 (orange) after illumination with saturating red light was observed at 750 nm. For reference, the dashed green and orange lines denote the absorbance at 750 nm in the far-red illuminated spectra of DmF1 and DrF1 (see panels a, b). e When incubated in darkness (‘D’) in the presence of ATP, both DmF1 and DrF1 trigger an increase in phosphorylated FixJ (p-FixJ) as visualized by slower migration in a Phos-tag gel than the unphosphorylated form. By contrast, under red light (‘R’), FixJ remains unphosphorylated, as it does if no SHK is included in the reaction (‘-‘). Experiments were repeated three times with similar outcomes. f DmF1 and DrF1 were incubated in the presence of FixJ and a double-stranded DNA molecule containing the operator site for phospho-FixJ and a 5′-attached TAMRA fluorescence label (see Suppl. Fig. 6a). Following illumination with far-red light (to populate the Pr state, indicated by purple bar), the reaction was initiated by ATP addition, and the TAMRA fluorescence anisotropy was monitored over time. The signal rise indicates formation of phospho-FixJ and binding to the DNA. Illumination with red light (marked in red) leads to a decrease in signal, indicative of net phosphatase activity. Experiments were done in triplicate (n = 3) with similar outcomes (see Suppl. Fig. 6b, c). Source data are provided as a Source Data file.
The Pfr state may also revert to Pr thermally in the so-called dark recovery reaction. As for instance evidenced in certain blue-light receptors23,35,36, changes in the dark-recovery kinetics can translate into sizeable sensitivity differences under prolonged illumination. Put simply, if a given photoreceptor recovers to its dark-adapted state much faster than another receptor, it will take proportionally more light to populate the light-adapted signaling state to the same degree at photostationary state32,37. To investigate potential differences in dark recovery, DrF1 and DmF1 were illuminated with red light, and the recovery to the Pr state was monitored over time at 25 °C by tracking A750 (Fig. 2d). In line with earlier measurements on BphPs38, the recovery was slow and incomplete even after 24 h for both receptors (Suppl. Figs. 4c and 5). Over the initial 20 h, the recovery proceeded with similar kinetics in DmF1 (0.098 h−1) and DrF1 (0.059 h−1), but the amplitude was markedly higher in case of DmF1 (66% versus 10%), leading to faster overall dark reversion (Fig. 2d, Suppl. Fig. 5). Longer measurements were precluded because of the onset of protein aggregation. Given its slow kinetics, we expect the dark recovery to have a minor effect on the pREDusk and pREDawn reporter-gene experiments. If anything, the slower and less complete recovery of DrF1 should translate into a higher light sensitivity at photostationary state for DrREDusk relative to DmREDusk but the opposite is the case. Taken together, we therefore conclude that the photochemical properties of DmF1 and DrF1 do not underlie the observed sensitivity difference.
Molecular basis of enhanced light sensitivity
We suspected that the diverging dose-response profiles of DmREDusk and DrREDusk might be rooted in the enzymatic activities of their underlying DmF1 and DrF1 SHKs under different light conditions. Thus, we assayed the phosphotransfer activity of DmF1 and DrF1 to the FixJ RR using Phos-tag assays10. Briefly, these experiments resort to polyacrylamide gels containing a chelating agent that lowers the electrophoretic migration speed of phosphorylated proteins relative to their non-phosphorylated forms. Upon ATP addition, both DmF1 and DrF1 promoted the phosphorylation of FixJ in darkness but not under red light (Fig. 2e). These observations agree with the pREDusk reporter-gene assays and indicate that both DmF1 and DrF1 act as net kinases in darkness. That is, their elementary kinase activity outweighs their phosphatase activity (if present) under these conditions.
To get quantitative insight into these elementary activities of DmF1 and DrF1, we next adapted a histidine-kinase assay pioneered for the blue-light-responsive SHK YF123,39. In brief, the assay employs a double-stranded DNA fragment that harbors the binding site for phospho-FixJ and is labeled with tetramethylrhodamine (TAMRA). Once phosphorylated by an SHK, FixJ binds to the DNA, thereby incurring a decrease in rotational diffusion and a concomitant increase of TAMRA fluorescence anisotropy. In darkness, DmF1 and DrF1 prompted anisotropy increases of 0.08 h−1 and 0.11 h−1, respectively, upon ATP addition, indicative of FixJ phosphorylation (Fig. 2f, Suppl. Fig. 6). Consistent with the reporter-gene experiments and the Phos-tag assays, these data reflect that both receptors act as net kinases in darkness (i.e., in the Pr state). Analogous to YF123,39, exposure to red light switched the activity to that of a net phosphatase, and the anisotropy signal dropped. Intriguingly, the phosphatase activity was markedly stronger in DmF1 with an anisotropy decrease at a rate of −0.30 h−1 compared to DrF1 with a rate of −0.06 h−1 which immediately accounts for the different light sensitivities of DmREDusk and DrREDusk. That is because the phosphatase activity of DmF1 under red light (in its Pfr state) greatly outweighs its kinase activity in darkness (in its Pr state). Consequently, only a relatively small fraction of DmF1 needs to be converted to Pfr to tilt the kinase-phosphatase balance to net phosphatase at the ensemble level. Ergo, less red light is required for actuation of the DmREDusk circuit than for DrREDusk (see Fig. 1c).
Linker modifications as a means of reprogramming two-component output
The above experiments showcase that modulation of the kinase-phosphatase duality can greatly impact on SHK activity and TCS output. Spurred by these findings, we explored modifications of the linkers connecting the PSM and DHp/CA moieties of the SHKs. As noted above, said linkers commonly adopt α-helical coiled-coil structures within the homodimeric SHK, which was recently confirmed for the DrBphP by cryo-electron microscopy (Suppl. Fig. 1)20. These linkers serve as crucial conduits in signal transduction, and alterations of their length and sequence suffice to utterly alter the kinase-phosphatase equilibrium and thereby reprogram TCS signal output27,40,41. We thus applied the PATCHY method27 to generate a library of DmF1 variants with their linkers extended or shortened by up to 40 residues. As detailed before27, PATCHY uses PCR amplification with sets of staggered forward and reverse primers to produce SHK variants differing in the length and sequence of their linkers connecting the BphP PSM and histidine-kinase effector moieties (Suppl. Fig. 7a). Importantly, the linker sequences are not varied at random but derive from the linkers of the parental receptors, that is, DmBphP and B. japonicum FixL in the case of DmF1. PATCHY library generation was conducted within the DmREDusk context, thus enabling the ready screening under dark and red-light conditions for light-responsive variants. Doing so identified six unique variants that exhibited light responses similar to DmREDusk, i.e., greater reporter-gene expression in darkness than under red light (Fig. 3a, Suppl. Fig. 7b). We refer to these variants by their linkers lengths relative to DmF1. For instance, the variant DmF1-4 has a linker four residues shorter than that of DmF1. Pairs of certain variants had the same length of the linker but different sequences, which we designate by the suffixes ‘a’ and ‘b’, e.g., in the case of the variants DmF1-18a and DmF1-18b (Fig. 3a, Suppl. Fig. 7b). Most variants exhibiting light-repressed reporter expression possess linkers longer than that in DmF1 by up to 38 residues (Fig. 3b). Given the coiled-coil nature of the linker with two α-helical turns per seven residues, we also analyzed the linker lengths in terms of their heptad periodicity (Fig. 3c)27. Strikingly, most variants populated a heptad register shifted by +3 residues relative to DmF1. That is, their linkers were extended or shortened by ±7 × n + 3 residues compared to DmF1, where n is an integer number.
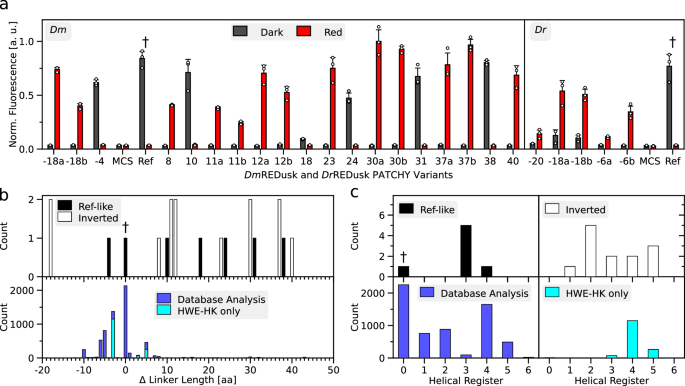
a Bacteria harboring different DmREDusk (left) and DrREDusk (right) systems were incubated under red light (red bars) or in darkness (gray). The DsRed reporter fluorescence was determined as the mean ± s.d. of n = 3 biologically independent replicates and compared to that of DmREDusk, DrREDusk (both denoted ‘Ref’ and labeled with †), and of controls lacking the fluorescent protein but containing a multiple-cloning site (MCS) instead. The relative length of the linker connecting the photosensory module (PSM) and the effector moiety of the SHK variants is indicated on the abscissa. Variants with identical linker lengths but different sequences are designated with the suffixes ‘a’ and ‘b’. The actual linker sequences are provided in Suppl. Fig. 7b. b Distribution of relative linker lengths of DmREDusk variants (top panel), with red-light-repressed (termed ‘Ref-like’) and inverted, red-light-activated specimens (termed ‘Inverted’) shown as black and white bars, respectively. The dagger symbol (†) denotes the linker length in DmF1 that underlies the original DmREDusk. The bottom panel shows in comparison the linkers in naturally occurring BphP SHKs. Separate analyses consider all histidine-kinase families (blue) or only HWE SHKs (cyan). c Analysis of the linker lengths in panel b according to their heptad coiled-coil register. To this end, the distribution is plotted against the remainder after division by seven of the linker length (that is, the modulo 7). Color codes as in panel b. Source data are provided as a Source Data file.
Intriguingly, the PATCHY library also contained 13 unique DmREDusk variants with an inverted response to red light, i.e., they prompted higher reporter expression under red light than in darkness (Fig. 3a, Suppl. Fig. 7b). Whereas two of the inverted variants had linkers that are 18 residues shorter than in DmF1, the remainder exhibited more extended linkers. In contrast to the above light-repressed variants, the linker lengths of the inverted, light-activated variants did not concentrate on a single heptad register but spanned several. We extended our analyses to the DrREDusk context and, by again applying PATCHY, identified five unique variants affording red-light-activated gene expression (Fig. 3a, Suppl. Fig. 7b). All these variants possessed shorter linkers than the parental DrF1 and fell into the linker registers +1 and +3. The broad distribution of linker registers in the light-activated DmF1 and DrF1 variants contrasts with that observed for YF1 where signal-inverted, light-activated variants essentially fell into the helical register +1 relative to the original, light-repressed SHK23,27.
We wondered whether the predominance of certain linker lengths and registers among the DmF1 and DrF1 variants identified by PATCHY correlates with the lengths of such linkers in naturally occurring BphP-coupled SHKs. A database analysis (Fig. 3b) revealed that the lengths of these linkers clustered within around ±10 residues relative to the parent DmF1 and DrF1 receptors. The linkers populated several heptad registers, merely the +3 and +6 registers were notably underrepresented (Fig. 3c). Interestingly, the +3 linker register into which the majority of light-repressed DmF1 variants fall is thus but poorly represented among the natural BphP-coupled SHKs. The principal analysis results were similar when excluding HWE histidine kinases that may employ different signal-transduction mechanisms42,43. Taken together, the linkers presently identified by PATCHY therefore cover regimes that are only infrequently, if at all, used in nature. Whereas the underlying reasons for the comparatively narrow linker length distribution in the natural receptors remain unclear, at the very least our data indicate that linkers outside the natural parameter space can principally support intact SHK signal transduction as well.
Inverted two-component systems facilitate optogenetics and biotechnology
Inversion of the response to light is not only mechanistically interesting, but also it provides opportunities for applications in biotechnology and optogenetics32. Based on particularly stringent regulatory responses, we subsequently focused on DmF1 and DrF1 variants with linkers 23 residues longer and 6 residues shorter, respectively, than their parental systems (denoted as ‘23’ and ‘−6b’ in Fig. 3a and Suppl. Fig. 7b), and we refer to these variants as DmF1+23 and DrF1-6b. Given their inverted light responses, the associated optogenetic circuits are designated as DmDERusk and DrDERusk in the following. Both circuits afford qualitatively similar responses as DmREDawn and DrREDawn, i.e., induction of expression by red light (Suppl. Fig. 3), but do away with the λ phage cI repressor. Removal of this repressor offers at least two advantages: first, it reduces the metabolic burden on the bacteria; second, it facilitates the combination with genetic circuits that rely on this repressor. Given these benefits, we characterized in detail the response to red light of bacteria harboring the DmDERusk and DrDERusk systems (Fig. 4a, b). In case of DmDERusk, the DsRed reporter fluorescence was low in darkness but monotonically increased with red light by 300-fold and with a half-maximal light dose of (2.0 ± 0.1) µW cm−2. For DrDERusk, the reporter fluorescence increased around 90-fold under red light with a half-maximal dose of (0.60 ± 0.04) µW cm−2 (Fig. 4a). Perplexingly, the DrDERusk circuit based on the DrPSM thus afforded higher light sensitivity than DmDERusk based on the DmPSM, which contrasts with the observations made for DrREDusk vs. DmREDusk (see Fig. 1c). Flow cytometry revealed uniformly low reporter fluorescence in darkness for DmDERusk and DrDERusk that was 1.5-fold and 1.8-fold, respectively, above the background level determined for an empty-vector control (MCS) (Fig. 4b). Red light induced a uniform shift of the single-cell fluorescence distribution to 70-fold and 51-fold higher values, respectively. Thus, both systems have low basal expression (i.e., leakiness), and support stringent regulation by red light in an essentially all-or-none manner.
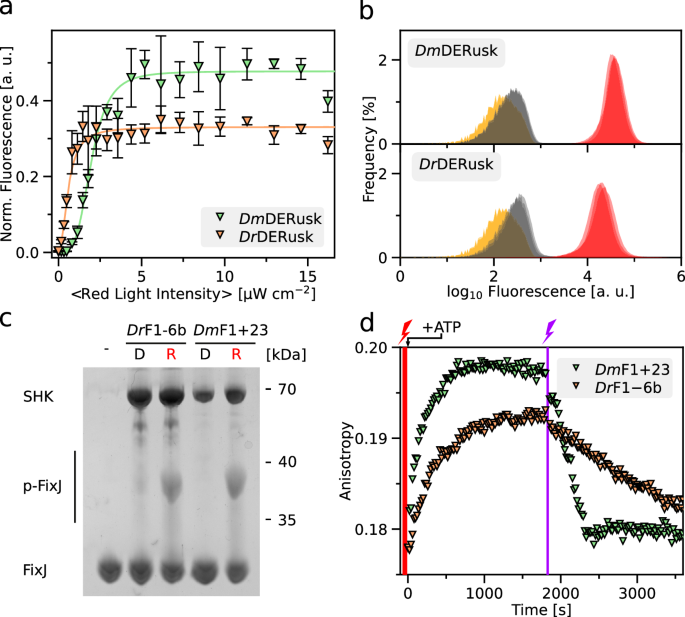
a DsRed production of bacteria harboring DmDERusk (light green triangles) or DrDERusk (light orange triangles) as a function of red-light intensity. Fluorescence readings were normalized to the optical density of the bacterial cultures at 600 nm (OD600) and corrected for background fluorescence. Data represent mean ± s.d. of n = 3 biologically independent replicates and were normalized to DmREDusk in darkness. Light intensities are averaged over the duty cycle as marked by angled brackets. b Analysis by flow cytometry of bacteria carrying DmDERusk (top) or DrDERusk (bottom) under red light (red) or in darkness (gray). The yellow curves denote control bacteria harboring empty vectors with a multiple-cloning site replacing DsRed. n = 3 biologically independent replicates for each sample are displayed. c The sensor histidine kinases (SHK) DmF1+23 and DrF1-6b, underpinning DmDERusk and DrDERusk, respectively, were analyzed on Phos-tag gels as described in Fig. 2e. Both SHKs promote the phosphorylation of the response regulator FixJ under red light compared to darkness, consistent with the reporter-gene data shown in panels a and b. d The enzymatic activity of the DmF1+23 and DrF1-6b SHKs was also monitored by fluorescence anisotropy (see Fig. 2f, Suppl. Fig. 9a). Illuminated with red light (indicated by red flash), both variants exhibit net kinase activity and phosphorylate FixJ, as revealed by rising TAMRA fluorescence anisotropy. Exposure to far-red light (purple flash) converts both DmF1+23 and DrF1-6b to net phosphatases that promote the dephosphorylation of FixJ, as reflected in decreasing fluorescence anisotropy. Experiments were done in triplicate (n = 3) with similar outcomes (see Suppl. Fig. 9b, c). Source data are provided as a Source Data file.
To unravel the molecular basis for the unexpected higher light sensitivity in DrDERusk compared to DmDERusk, we expressed and purified the underlying SHKs DrF1-6b and DmF1+23. Absorbance spectroscopy confirmed BV chromophore incorporation and light-driven Pr⇄Pfr interconversion as in the parental DrF1 and DmF1 SHKs (Suppl. Fig. 8). Notably, DrF1-6b and DmF1+23 incorporated BV to comparable extents and underwent Pr→Pfr conversion under red light with closely similar speed and efficiency (Suppl. Fig. 8). We next assessed the enzymatic activity of these SHKs in darkness and under red light by Phos-tag analysis (Fig. 4c). Consistent with their inverted response within the reporter-gene context (see Fig. 4a), both DrF1-6b and DmF1+23 showed responses to light opposite those seen for DrF1 and DmF1 (see Fig. 2e) in that substantial FixJ phosphorylation occurred under red light but not in darkness. To obtain quantitative insight, we assessed the enzymatic activity and light response of these SHKs by fluorescence anisotropy (Fig. 4d, Suppl. Fig. 9). Under red light, both DrF1-6b and DmF1+23 exhibited net kinase activity with anisotropy increases of around 0.14 h−1 and 0.18 h−1, respectively. Exposure to far-red light converted both variants to net phosphatases. Notably, the phosphatase activity of DmF1+23 (fluorescence anisotropy decrease of −0.12 h−1) trumped that of DrF1-6b (−0.02 h−1) which can account for the differing light sensitivities of DmDERusk compared to DrDERusk. Given the comparatively weak phosphatase activity of DrF1-6b, red-light-induced Pr→Pfr conversion of a relatively small SHK portion suffices for net kinase activity at the ensemble level. In the case of DmF1+23, the phosphatase activity in the dark-adapted state is stronger, and consequently a larger SHK fraction needs to be converted to the Pfr state to result in net kinase activity.
We next addressed whether these systems support multiplexing with optogenetic circuits that drive gene expression in response to other light colors. We previously combined DrREDusk and DrREDawn with the blue-light-responsive pCrepusculo and pAurora circuits28,44,45 (Suppl. Fig. 10a). Briefly, the latter two setups rely on the light-oxygen-voltage receptor PAL46 that sequence-specifically binds a small RNA molecule, termed aptamer, once triggered by blue light. In pCrespusculo45, this aptamer is interwoven with the ribosome-binding site of a target gene, and thereby PAL represses the translation and expression of this gene under blue light. pAurora inverts and amplifies the response to blue light by including a gene-inversion cassette based on the λ phage cI repressor (Suppl. Fig. 10a). Given that pAurora and DrREDawn share the cI component, crosstalk is fully expected, irrespective of these circuits responding to different light colors. To demonstrate this aspect, we combined in the same bacterial cells a pAurora plasmid driving DsRed expression and a DrREDawn plasmid controlling the expression of the yellow-fluorescent YPet protein (Suppl. Fig. 10b, c). Said bacteria were exposed to combinations of blue and red light, and the DsRed and YPet fluorescence was measured. Compared to darkness, both fluorescence readings monotonically increased with the doses of blue and red light. Owing to the crosstalk, the DsRed and YPet fluorescence rose in lockstep, and the maximum values were only reached in the presence of both blue and red light. While potentially of utility in some applications, commonly crosstalk is undesired. When combining pAurora-DsRed with DmDERusk-YPet (Fig. 5a), the crosstalk was eliminated, and the circuits could be induced by blue and red light, respectively, largely independently of each other (Fig. 5b, c).
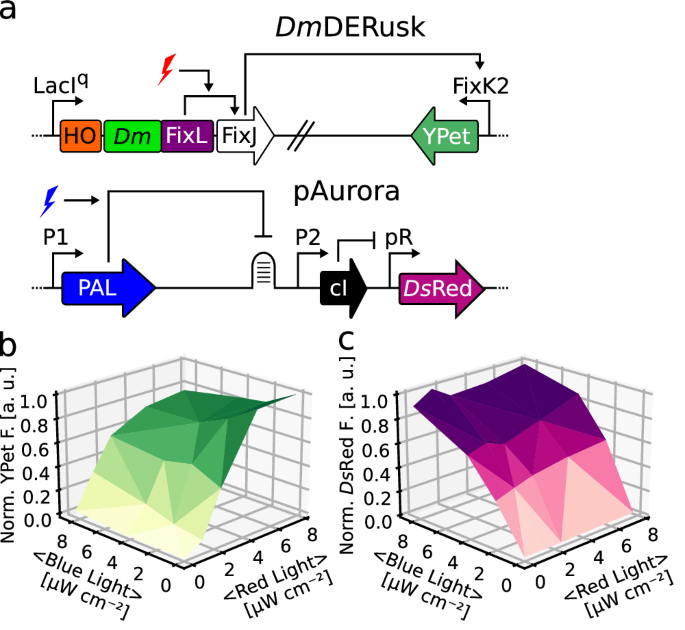
a Schematics of the red-light-responsive DmDERusk circuit, developed at present, and of the earlier pAurora system that reacts to blue light45. b, c Bacteria harboring both pAurora-DsRed and DmDERusk-YPet were incubated under different red and blue light intensities. The YPet (b, green) and DsRed (c, pink) fluorescence readings were normalized to the optical density of the bacterial cultures at 600 nm (OD600) and corrected for background fluorescence. Data represent the mean of n = 3 biologically independent replicates. Light intensities are averaged over the duty cycle as marked by angled brackets. Source data are provided as a Source Data file.