In silico analyses of PcPAL towards β-MeCA
Currently, the E1cb (via a carbanion intermediate) and the E2 (concerted) mechanisms are widely accepted for PcPAL-mediated deamination reactions27. Consequently, the amination reactions catalyzed by PcPAL are likely to proceed through the reverse E1cb and E2 mechanisms (Fig. S1). Despite an ongoing debate between these mechanisms, both of them involve two critical steps in the amination reactions: amination and protonation. The key residues involved in these processes include the MIO-NH2 adduct, Tyr110, and R354. Among them, the MIO-NH2 adduct is responsible for amidating the αC of the substrate, Tyr110 is responsible for protonating the βC, and R354 forms strong hydrogen bonds with the carboxyl group of the substrate to position the substrate. Based on these catalytic processes, we conducted a series of in silico analyses to elucidate the underlying reasons for the inability of PALs to recognize β-substituted substrates. Firstly, CA and β-MeCA were individually docked into PcPAL (PDB:6HQF)52 (Fig. S2a), and based on the docking results, we further performed conventional molecular dynamics (cMD) simulations. The results showed that in the (β-MeCA)/(PcPAL-WT) complex, the distance variation range between the N atom of MIO and the αC of β-MeCA was narrower than that in the (CA)/(PcPAL-WT) complex (Figs. S3a, b). Besides, by examining the hydrogen bond interactions between the substrates and amino acids within the active pocket (Source Data 3), it was found that in the (β-MeCA)/(PcPAL-WT) complex, the carboxyl group of β-MeCA forms a high-frequency hydrogen bond with Tyr110, appearing in 99% of the recorded 5000 snapshots (Source Data 3). These evidences suggest that β-MeCA is more stable within the pocket. Although the reason for the inactivity of the (β-MeCA)/(PcPAL-WT) complex remains unclear, based on the above observations, we reasonably speculate that the inactivity is likely due to the perturbation of the substrate positioning dynamics53,54.
To validate our hypothesis, we further carried out umbrella sampling (US) simulations to determine the optimal amination distances (distance 1 in Fig. S4) when CA and β-MeCA have the lowest energy conformations in PcPAL-WT. For substrate CA, the optimal amination distance ranged from 3.5 to 3.9 Å when it had the lowest energy conformations in PcPAL-WT, whereas the corresponding distance ranged from 3.6 to 3.7 Å for β-MeCA (Fig. S2b). Besides these different distance ranges detected in the presence of CA and β-MeCA, we also observed differences related to the angle between MIO and the double bond of the substrates, which is important for the amination process. Specifically, we observed two angles in the (CA)/(PcPAL-WT) complex (70° and 109°, Fig. 2a). However, in the (β-MeCA)/(PcPAL-WT) complex, only one angle was detected around 80° (Fig. 2b). According to the Bürgi-Dunitz angle theory55, the N atom of MIO should attack from the direction of 105 ± 5° relative to the C=C bond to achieve effective orbital overlap for C-N bond formation. This missing bond-forming angle, along with the relatively narrow optimal amination distance range detected in the (β-MeCA)/(PcPAL-WT) complex, suggests that β-MeCA is less flexible and more stable than CA. Further, we compared the pocket volumes in the presence of both CA and β-MeCA. The results showed that the mean pocket volumes of the (CA)/(PcPAL-WT) complex and the (β-MeCA)/(PcPAL-WT) complex were 192.8 Å3 and 177.4 Å3, respectively (Fig. 2c, d). The smaller pocket volume within the (β-MeCA)/(PcPAL-WT) complex indicates that β-MeCA was confined to a very narrow cavity, which restricts its dynamic changes. Overall, all the US simulation results observed in the (β-MeCA)/(PcPAL-WT) complex indicated that the presence of β-methyl substitution may disrupt the normal substrate positioning dynamic.
a, b Gibbs energy diagram of the cMD simulations projected onto the distance from the amino N of MIO to the αC of the substrates (X-axis) and the angle between MIO and the double bond of the substrates (Y-axis) within the (CA)/(PcPAL-WT) complex and the (β-MeCA)/(PcPAL-WT) complex, respectively. c, d the pocket volumes detected during the cMD simulations of (CA)/(PcPAL-WT) complex and the (β-MeCA)/(PcPAL-WT) complex, respectively. e, f Optimized pre-reaction states of CA and β-MeCA in the PcPAL-WT using the Quantum Mechanics/Molecular Mechanics (QM/MM) methodology, respectively. Source data are provided in Source Data 3 to Source Data 5. CA: cinnamic acid; β-MeCA: β-methyl cinnamic acid; MIO: 3,5-dihydro-5-methylidene-4H-imidazol-4-one.
Following, Quantum Mechanics/Molecular Mechanics (QM/MM) simulations were employed to further optimize the conformations of PcPAL-WT and the substrate complexes. The QM/MM simulation results showed that, compared to the CA substrate (Fig. 2e) a strong hydrogen bond was detected between the hydroxyl hydrogen of Tyr110 and the carboxyl group of β-MeCA (1.51 Å, Fig. 2f). The formation of this strong hydrogen bond is thermodynamically unfavorable in the enzymatic reaction of PcPAL-WT, not only restricting the flexibility of β-MeCA in the active pocket but also significantly impeding the proton transfer process from Tyr110 to the βC-carbon atom.
In summary, our computational simulations indicated that the incapacity of PcPAL-WT to aminate β-MeCA raised from the perturbation of β-MeCA’s positioning dynamic within the active pocket, which is primarily due to the strong hydrogen bond formation between the carboxyl group of β-MeCA and Tyr110. Consequently, we hypothesized that alleviating the stringent active pocket of PcPAL might help restore the correct positioning dynamic of β-MeCA analogs to achieve the asymmetric amination for the synthesis of β-branched amino acids. Besides, our computational results indicated that to induce reactive conformations, it is essential to engineer not only the residues around the β-methyl group of β-MeCA, but also those around its phenyl ring. This is crucial as both sets of residues were essential to form intricate interactions with β-MeCA and influence its conformation in the active pocket.
Rational engineering of PcPAL for the amination of β-MeCA
As the above computational results provided us with understandings for the rational engineering of PcPAL, we identified eight key residues around the substrate binding pocket (L134, F137, L138, I460, E484, L256, N260, and L206), which could affect the correct conformation of β-MeCA (Fig. 3a). Among them, L256 and N260 are located near the β-Me group, while the others are around the phenyl ring of β-MeCA. Subsequently, each of these residues was individually substituted with three smaller residues (A, G, and V). Additionally, we also investigated three other mutants, previously established in our laboratory (E484D, F137V-I460V43, and F137A-I460A43) to explore their potential. Notably, the latter two mutants, developed by the Bencze’s group43, were investigated for their ability to catalyze the amination of bulky substrates, such as styrylacrylate and biarylalanines. After recombinant expression in E. coli BL21 (DE3) and purification of these 27 mutants, an in vitro screening against β-MeCA was performed. Remarkably, after reacting with three mutants, i.e., L256V, I460V, and E484D, the product concentrations in the reaction system achieved 24.3%, 8.7%, and 5.3%, respectively. Interestingly, L256V and I460V mutants were well studied previously for synthesizing various l-phenylalanine analogs43,44,45,56,57, yet their ability to convert β-substituted CA analogs was not explored yet. Through analyzing the conformation of β-MeCA within the docking result, we found that L256 is positioned in close proximity to the β-methyl group of the substrate (Fig. 3a). After substitution with Val, more space becomes available to accommodate the β-methyl group. This is a plausible explanation for the significantly higher activity exhibited by the L256V mutant. I460 and E484 are located around the phenyl group of β-MeCA. After turning them into smaller residues, we thought that the substrate might undergo spatial displacement within the active pocket. This should lead to the generation of additional conformations, among which favorable ones for the reaction may be present, thereby demonstrating reaction activity. To find much better performing variants, we further combined these promising mutants to generate four additional mutants (L256V-I460V, L256V-E484D, I460V-E484D, and L256V-I460V-E484D). Among them, the L256V-I460V mutant showed the best amination activity with a product concentration in the reaction system of 49.1% (Fig. 3b). Contrary to our initial expectations, the double mutant L256V-I460V showed much better activity than the triple mutant L256V-I460V-E484D (product concentrations in the reaction system: 7.2%), indicating that residues at positions 256 and 460 were the most crucial mutation sites. Additionally, it is intriguing to note that when the E484D mutation was combined with other mutations, it consistently led to a decrease in the activity of the original mutants, which indicated that the multi-point mutation combined with E484D seems to be unfavorable to ensure a correct binding position of β-MeCA in the active site pocket.
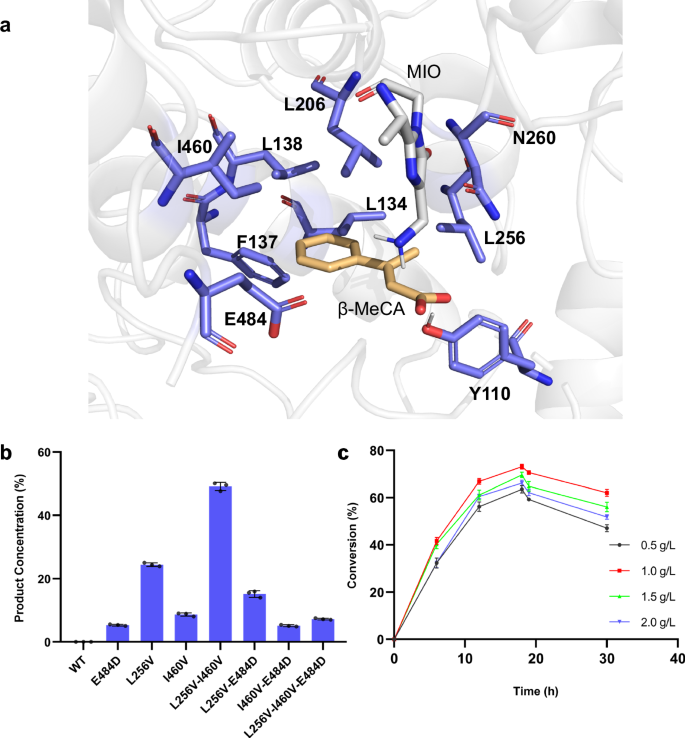
a Key bulky residues around the substrate β-MeCA modeled in PcPAL-WT. The active site illustrations are based on the crystal structure of PcPAL (Protein Data Bank ID: 6HQF). The substrate and MIO group are highlighted in orange and gray, respectively. b Enzyme activity of PcPAL mutants towards β-MeCA. Reaction conditions: 100 µg purified enzyme, 1 mM β-MeCA, total 200 µl in NH4OH buffer (pH 10.0), 30 °C, 450 rpm for 24 h. After that the reaction was stopped by adding HCl and centrifuged for further HPLC analysis. c Time curves with different substrate concentrations. Three parallel samples were collected from each reaction for HPLC analysis. Product concentrations were calculated using a standard curve and the peak area of the product. Error bars (SD) were derived from triplicate experiments and created using GraphPad Prism 8. Data are presented as mean values ± SD. Source data are provided in Source Data 1. β-MeCA: β-methyl cinnamic acid; MIO: 3,5-dihydro-5-methylidene-4H-imidazol-4-one.
To further evaluate the catalytic efficiency of the PcPAL-L256V-I460V mutant, we measured its kinetic constants in the deamination reaction using (2S, 3R)-β-MePhe as substrate (Fig. S6). The results revealed a relatively high Km value (Km = 0.7304 ± 0.1195 mM), which indicated the low affinity of (2S, 3R)-β-MePhe to the mutant. This weak affinity allows the product to leave the catalytic pocket more easily during the amination reaction, thereby facilitating the amination reaction.
Determination of the absolute configuration of the amination product
Encouraged by the above findings, we elucidated the absolute configuration of the amination products by employing two distinct methods. In the first method, by utilizing the three β-methyl phenylalanine (β-MePhe) standards with 2S,3S-, 2S,3R-, 2R,3R– configurations, the in vitro deamination reactions were conducted using the most efficient variant (PcPAL-L256V-I460V). High-performance liquid chromatography (HPLC) analysis demonstrated that only (2S,3R)-β-MePhe was deaminated with full (~100%) conversion (Fig. 4a). Given the reversible nature of PAL-mediated reactions, we deduced that the stereo configuration of the amination product should hence be 2S,3R. To further validate our hypothesis, we conducted the second approach, in which Marfey’s reagent was used to derivatize the above three β-MePhe standards as well as the crude amination reaction solution which was formed by the PcPAL-L256V-I460V mutant. As (2R, 3S)-β-MePhe was not commercially available, the β-MePhe mixture standard which contains all four configurations was chosen for the derivatization reaction. After analyzing this by LC-HRMS, we detected the derivatization product of (2R, 3S)-β-MePhe (blue peak in Fig. 4b viii), confirming the presence of the (2R, 3S)-typed substrate in the standard mixture. Moreover, we found that when the crude amination reaction solution was reacted with Marfey’s reagent, a peak with the same retention time was observed as the one in the (2S, 3R)-type standard (Fig. 4b and Fig. S7). Hence, the results from both experiments clearly confirmed that the absolute configuration of the amination product was 2S, 3R. Furthermore, the amination reaction catalyzed by the PcPAL-L256V-I460V mutant proceeded with excellent diastereoselectivity (dr > 20:1) and enantioselectivity (ee > 99.5%).
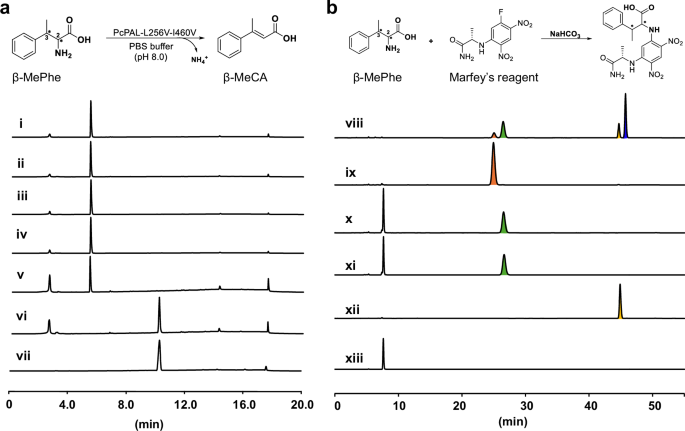
a In vitro deamination assays with β-MePhe of different absolute configurations using the PcPAL-L256V-I460V mutant. Reaction conditions: 100 µg purified enzyme, 1 mM various β-MePhe, total 500 µL in PBS buffer (pH 8.0), 30 °C, 450 rpm for 24 h. i) (2S, 3S)-β-MePhe; ii) deamination reaction of (2S, 3S)-β-MePhe; iii) (2 R, 3R)-β-MePhe; iv) deamination reaction of (2R, 3R)-β-MePhe; v) (2S, 3R)-β-MePhe; vi) deamination reaction of (2S, 3R)-β-MePhe; vii) β-MeCA standard. b Derivatization results with β-MePhe of different configurations and the in vitro reaction product formed by using Marfey’s reagent. viii) standard mixture of β-MePhe derivatized with Marfey’s reagent; ix) (2S, 3S)-β-MePhe derivatized with Marfey’s reagent; x) (2S, 3R)-β-MePhe derivatized with Marfey’s reagent; xi) derivation of the in vitro amination reaction product produced by the PcPAL-L256V-I460V mutant; xii) (2R, 3R)-β-MePhe derivatized with Marfey’s reagent; xiii) blank containing solely Marfey’s reagent and enzyme. β-MePhe β-methyl phenylalanine, β-MeCA β-methyl cinnamic acid.
Development of a whole-cell biotransformation process
To enhance the practicality of this direct asymmetric amination reaction for synthesizing β-branched aromatic amino acids, we developed a whole-cell biotransformation (Fig. 1c)44, 56. Therefore, the gene of the PcPAL-L256V-I460V mutant was introduced into the strain E. coli BL21 (DE3)_ΔtyrB, which lacks the aromatic amino acid transaminase gene tyrB and thus avoids potential transamination of the generated product within the cells58. After expressing the PcPAL-L256V-I460V mutant at 28 °C for 20 h, the cells were harvested and then resuspended in ammonia buffer for the biotransformation. To make this process efficient, we optimized different conditions by using β-MeCA as substrate and a commonly used 5 M ammonium hydroxide buffer (NH4OH buffer, pH 10) as the source of ammonia48,50. Considering the possible membrane permeability issues, clarified lysate of the harvested cells was used for transformation. However, the results showed that employing whole-cells containing the PcPAL-L256V-I460V gave significantly higher conversions than using clarified lysate, presumably due to higher enzyme stability in vivo under the same catalytic conditions (Fig. S8). Moreover, by resuspending the cultivated cells in NH4OH buffer to reach an OD600 of 30, four distinct substrate concentrations (0.5, 1.0, 1.5, and 2.0 g/L) were systematically investigated. The maximum product concentrations in the reaction system (71%) was achieved at a substrate concentration of 1.0 g/L after 18 h reaction time. Longer reaction times lead to a gradual decline indicating a potential shift in the reaction equilibrium towards the reverse deamination reaction (Fig. 3c). Optimal reaction conditions were thus identified using 5 M NH4OH buffer, 1 g/L substrate concentration, and 18 h reaction time.
Exploration of the substrate scope
Given the excellent stereoselectivity demonstrated by PcPAL-L256V-I460V, as well as the simple and practical whole-cell biotransformation method, we next explored the substrate scope of this amination reaction. 15 β-MeCA analogs with different substituents at the phenyl ring were firstly synthesized using a straightforward chemical method (Fig. S9)59. Considering that the amino acids around the phenyl ring of substrates in the active pocket of PcPAL, such as L134, F138, and F138, could also potentially influence the conformations of substrates, we conducted screening assays on the synthesized substrates using our mutant library. The HPLC results showed that six substrates (1, 2, 4 to 8) could be converted by the PcPAL-L256V-I460V mutant. Despite substrate 3, 9, and 10 could be converted by both PcPAL-F137V-I460V and PcPAL-L256V mutants, the combination mutant PcPAL-F137V-L256V-I460V showed higher conversion for these three substrates (Fig. S10), highlighting the crucial role of the L256 position in enhancing reaction activity.
Next, we scaled up the reactions using the E. coli based whole-cell biotransformation method by using 30 mg substrates and obtained the ten β-substituted amino acid products with yields ranging from 41% to 71% (Fig. 5). In previous reports, the absolute configuration of β-substituted amino acid products were typically determined by referencing to previously reported NMR and specific rotation data24. For the newly synthesized β-substituted amino acid products reported here, we assigned the absolute configurations for four products (4a, 5a, 8a, and 9a, Fig. 5b, Table S2 to S5) by X-ray crystallography and this unequivocally confirmed that the absolute configuration of all four crystallized products were 2S,3R, in accordance with the absolute configuration determined for β-MePhe 1a (Fig. 4). In addition, a comprehensive analysis of all the products was carried out, including NMR and specific rotation data analysis. Remarkably, all these analytical data consistently confirmed that the configurations of the produced β-branched phenylalanine analogs were 2S, 3R. All chiral reaction products were obtained with high diastereoselectivity (dr > 20:1) and optical purity (ee > 99.5%) (Fig. 5).
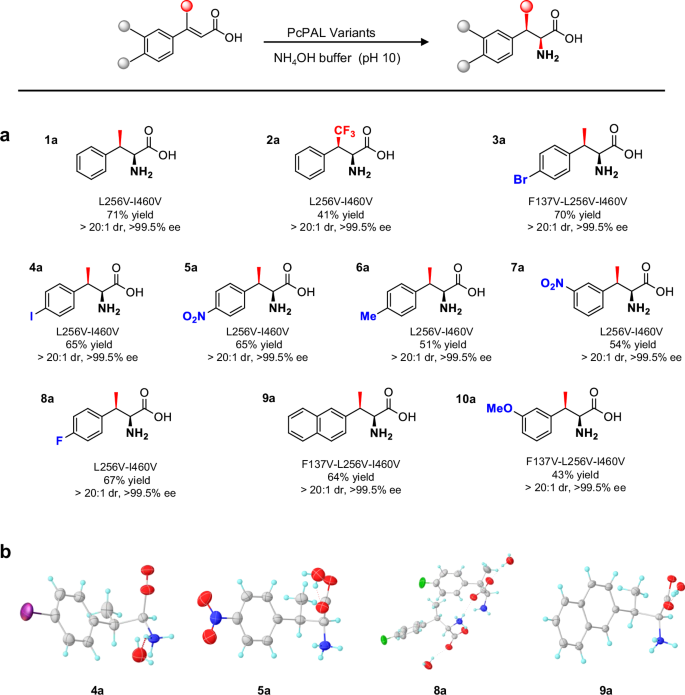
a Structures of the produced β-branched aromatic α-amino acids. Yields refer to the isolated product after purification. b X-ray crystal structures of products 4a, 5a, 8a and 9a. Different colors represent different atoms: gray for carbon atoms, cyan for hydrogen atoms, red for oxygen atoms, blue for nitrogen atoms, and green for fluorine atoms. Source data are provided in Source Data 5.
Next, by comparing the yields of these productive reactions, we identified three factors that could affect the amination reaction efficiency. Firstly, the position of substitutions plays a significant role. When the electronegativity of the substituents was the same, substrates with para substitutions (e.g., 3a, 4a, 5a, and 8a) exhibited higher reactivity compared to those with meta substitutions (e.g., p7). Notably, substrates with ortho substitutions, such as 11 and 12 (Fig. S9), were not converted. Secondly, the electronegativity of substituents could also influence the outcomes. A comparison of yields between products 3a–5a and 6a, as well as 7a and 10a, indicates that electron-withdrawing groups could benefit the conversion. Thirdly, the size of the substitutions at the β-position also plays a crucial role. Although mutants could accommodate the CF3 group at the β-position, which is similar in size to the methyl group, the yield of 2a remained relatively low (41 %). The introduction of this strong electron-withdrawing CF3 substituent should reduce the nucleophilicity of the α carbon, thus resulting in a slow amination process. We thought that this accounts for the diminished activity observed for the CF3-substituted substrate. However, the ability of mutants to recognize the CF3-substituted substrates strongly implies that steric hindrance also plays a central role in impeding the reaction. Conversely, when larger substitutions like ethyl, propyl, and isopropyl were present at the β-position (13 to 15, Fig. S9), no reaction was observed. This observation further underscores the high sensitivity of PcPALs’ active sites to steric effects at the β-position of the substrates.
Elucidating the molecular basis of PcPAL-L256V-I460V for converting β-MeCA
To reveal the molecular basis for accepting β-MeCA, as well as the excellent stereoselectivity during the PcPAL-L256V-I460V mediated amination reactions, the protein structure of this mutant was predicted using AlphaFold 2 and docked with β-MeCA into its active pocket. Subsequently, a series of computational simulations were conducted based on the docking results. Interestingly, similar simulation results were observed in the (β-MeCA)/(PcPAL-L256V-I460V) complex compared to the (CA)/(PcPAL-WT) complex. Specifically, in both complex systems, angles conforming to the Bürgi-Dunitz angle theory were detected, and both angles were around 109° (Figs. 6a and 2a). In addition, in the (β-MeCA)/(PcPAL-L256V-I460V) complex, the mean pocket volume of β-MeCA was around 207.9 Å3, much larger than that in the (β-MeCA)/(PcPAL-WT) complex (177.4 Å3, Figs. 6b and 2d), meaning that β-MeCA was more flexible in the mutant. Furthermore, the optimized conformation of the (β-MeCA)/(PcPAL-L256V-I460V) complex was determined through QM/MM simulations, revealing a distance of 3.28 Å between the hydroxyl hydrogen of Tyr110 and the carboxyl group of β-MeCA. This distance is greater than that from the hydroxyl hydrogen of Tyr110 to βC (2.11 Å) (Fig. 6c), with a corresponding hydrogen bond angle of 118.63° (Table S6, Source Data 5), indicating that the strong hydrogen bond interaction present in the (β-MeCA)/(PcPAL-WT) complex (Fig. 2f) does not exist in the mutant. Overall, the simulation results shown in Fig. 6a–c demonstrate that the conformation of β-MeCA in the mutant was almost same to that of CA in PcPAL-WT. Considering the conformation of CA in the PcPAL-WT, this could be used as a reference for assessing the potential occurrence of reactions when β-MeCA is used as a substrate. Indeed, the simulation results indicated that the positioning dynamic of β-MeCA was relatively flexible within PcPAL-L256V-I460V mutant, which could facilitate the amination reaction process.
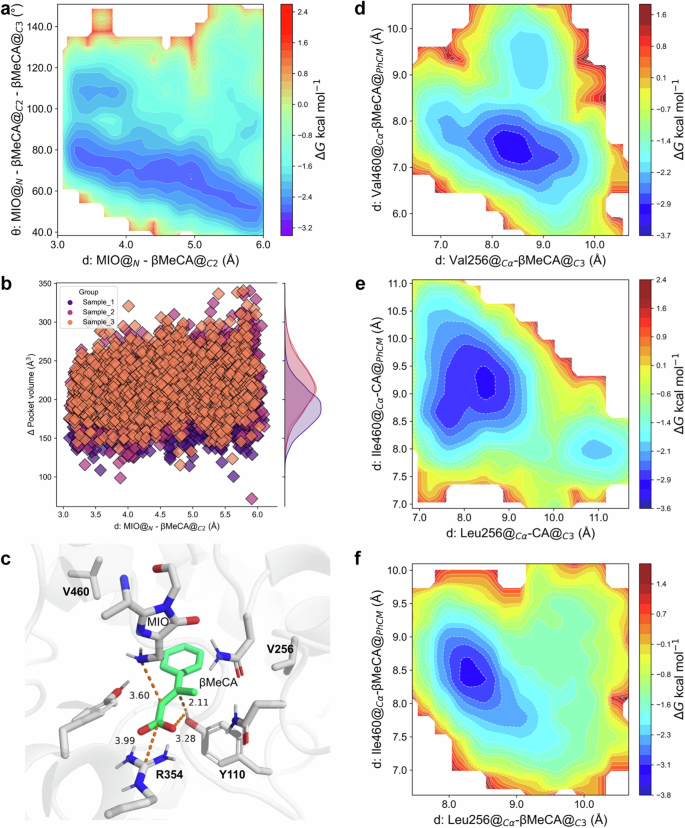
a Gibbs energy diagram of the cMD simulations projected onto the distance from the amino N of MIO to the αC of the substrates and the angle between MIO and the double bond of the substrates within the (β-MeCA)/(PcPAL-L256V-I460V) mutant. b the pocket volume detected during the US simulations of the (β-MeCA)/(PcPAL-L256V-I460V) complex. c Optimized pre-reaction states of β-MeCA in the PcPAL-L256V-I460V mutant using the Quantum Mechanics/Molecular Mechanics (QM/MM) methodology. d, e, f Gibbs energy diagram of the cMD simulations projected at the distance from βC of β-MeCA to amino acid 256 and the benzene ring center and amino acid 460 within the (β-MeCA)/(PcPAL-L256V-I460V) complex, (CA)/(PcPAL-WT) complex, and (β-MeCA)/(PcPAL-WT) complex respectively. Source data are provided in Source Data 3 to Source Data 5. CA cinnamic acid, β-MeCA β-methyl cinnamic acid, MIO 3,5-dihydro-5-methylidene-4H-imidazol-4-one.
To gain a deeper understanding of why β-MeCA showed different positioning dynamics within the PcPAL-WT and the PcPAL-L256V-I460V mutants, we further analyzed another two distances (distance 3: from βC of β-MeCA to amino acid 256; distance 4: from the benzene ring center of substrate to amino acid 460 in Fig. S4). In the complexes (β-MeCA)/(PcPAL-L256V-I460V) and (CA)/(PcPAL-WT), two distinct low-energy regions can be observed, each characterized by a broader primary energy well separated by a lower energy barrier (~1.2 kcal/mol), indicating that the substrate can readily traverse this barrier to adjust its conformation (Fig. 6d, e). Within the main low-energy regions, in the (β-MeCA)/(PcPAL-L256V-I460V) complex, distance 3 ranges from 7.9 to 8.6 Å, and distance 4 ranges from 8.0 to 8.9 Å (Fig. 6d). Similarly, in the (CA)/(PcPAL-WT) complex, distance 3 ranges from 7.4 to 8.9 Å, and distance 4 ranges from 8.3 to 9.8 Å (Fig. 6e). However, in the (β-MeCA)/(PcPAL-WT) complex, only one low-energy region is observed, with distance 3 ranging from 7.9 to 8.6 Å, and distance 4 ranging from 8.0 to 8.9 Å (Fig. 6f). These results indicate that compared to the (β-MeCA)/(PcPAL-WT) complex, β-MeCA in (PcPAL-L256V-I460V) exhibits a greater range of motion and a broader conformational space. Considering the wider angular range between MIO’s N atom and the double bond in (β-MeCA)/(PcPAL-L256V-I460V) and (CA)/(PcPAL-WT) complexes, these findings support our hypothesis that substrate positioning dynamics influence the occurrence of the reaction.
Additionally, the unique and precise distribution of amino acids in the PcPAL’s substrate binding pocket determines the stereoselectivity of the asymmetric amination reaction. The amino acids around the benzene ring are mainly hydrophobic, while the ones around the carboxyl group are more hydrophilic (Fig. 6c). Guided by the unique distribution of amino acids within the pocket, the β-methyl group of β-MeCA demonstrates a preferential orientation towards the hydrophobic region. This will be advantageous for the enzyme’s adaptation and stereoselectivity towards β-MeCA analogs. Importantly, due to the fact that the critical MIO group is positioned on the si-si face of the substrate plane, the chiral center formed after the amino group attacking αC can only be 2S. Additionally, the crucial Tyr110 is located on the Re-Re face of the substrate plane. In such case, when the βC is protonated, the chiral center formed can only be 3 R. Overall, the distinctive amino acid distribution provides essential molecular foundations for correctly accommodating β-MeCA and facilitating a highly stereoselective asymmetric amination reaction.