Structural basis of CXCL12 and AMD3100 action on CXCR4
To stabilize active-state signaling complexes and improve protein yields, we made the following modifications to wild-type CXCR4: we replaced the N-terminal methionine with a hemagglutinin signal peptide22, included a previously characterized constitutively active substitution (N119S)23 and fused monomeric enhanced green fluorescent protein (GFP)24 and a FLAG tag to the receptor C terminus (Extended Data Fig. 1a). We refer to this construct as CXCR4EM. We also used a Gαi construct harboring dominant negative substitutions25 to facilitate the isolation of receptor–Gi complexes in the absence of stabilizing antibody fragments26. Fluorescence-detection size-exclusion chromatography (FSEC)27 experiments indeed indicated complex formation between CXCR4EM and Gi in the absence of agonist (Extended Data Fig. 1b). We prepared detergent-solubilized CXCR4EM–Gi complexes and first determined cryo-EM structures in apo, CXCL12-bound and AMD3100-bound states at overall resolutions of 2.7, 3.3, and 3.2 Å, respectively (Fig. 1, Extended Data Figs. 2 and 3, Table 1 and Supplementary Fig. 1). Each of the structures shows a prototypical arrangement of an active receptor coupled to a heterotrimeric G protein, including a hallmark kink of transmembrane helix 6 (TM6) relative to previously reported crystal structures of antagonist-bound CXCR4 (refs. 19,20) (Extended Data Fig. 4a). We, therefore, refer to the CXCR4 conformation in these structures as active.
a, Apo CXCR4–Gi complex. b, CXCR4–Gi–CXCL12 complex. Inset, the fit of the CXCL12 N-terminal tail (residues 1–10) in cryo-EM map, represented as a semitransparent surface. The locations of chemokine recognition sites 1 and 2 are labeled. The curved dotted line represents missing density for the distal N terminus of CXCR4, which has been reported to interact with CXCL12. The gray density on the extracellular side may correspond to a partially occupied second protomer of dimeric CXCL12. c, CXCR4–Gi–AMD3100 complex. Inset, the fit of the AMD3100 compound in cryo-EM map.
Our cryo-EM reconstruction of CXCR4EM–Gi–CXCL12 revealed a clear signal for the chemokine bound at the extracellular side of the receptor (Fig. 1a). Density for the chemokine N terminus (residues 1–12) was sufficiently resolved to build side chains (Fig. 1b), whereas the remainder of the ligand was less resolved because of flexibility and only permitted main-chain tracing (Extended Data Fig. 3i). Consequently, interactions between the chemokine N-terminal region and receptor orthosteric pocket (chemokine recognition site 2) were readily discernible, while interactions between the globular portion of the ligand and the N terminus of CXCR4 (ref. 28) (chemokine recognition site 1) were unclear. CXCL12 is known to exist in monomeric and dimeric forms that have been shown to yield distinct signaling outcomes upon CXCR4 binding29,30. A weak signal corresponding to a second protomer of the CXCL12 dimer could be observed in our cryo-EM reconstruction, consistent with the notion that dimeric forms of CXC ligands act on single receptor subunits20,31 (Extended Data Fig. 3i).
The binding mode of CXCL12 onto CXCR4 is overall similar to that found in published structures of CC and CXC chemokine–chemokine receptor complexes31,32,33,34,35,36 (Fig. 2 and Extended Data Fig. 4b). However, the CXCL12 binding pose observed in our structure notably differs from that of CXCL12 bound to atypical chemokine receptor 3 (ACKR3, formerly known as CXCR7)37, a promiscuous receptor that has been suggested to function as a chemokine ‘scavenger’ and has approximately tenfold higher affinity for CXCL12 than CXCR4 (refs. 38,39). The CXCL12 C-terminal α-helix is rotated approximately 70° when bound to ACKR3 relative to CXCR4 (Extended Data Fig. 4c). Correspondingly, the 40s loop of CXCL12 is situated proximal to the N-terminal region in CXCR4, while it is nearby extracellular loop 3 (ECL3) in ACKR3. In addition to the distinct overall chemokine–receptor docking orientations, the binding geometries of the CXCL12 N terminus within the orthosteric pockets of each receptor are unique (Extended Data Fig. 4d).
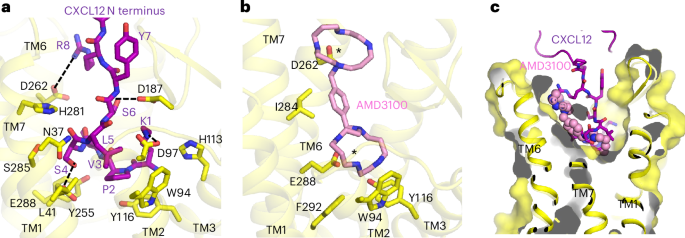
a, Expanded view of interaction between the CXCL12 N-terminal tail and CXCR4 orthosteric pocket. Hydrogen-bonding and electrostatic interactions are depicted as dashed lines. b, Expanded view of AMD3100 binding at CXCR4 orthosteric pocket. Asterisks indicate the positions of the two lactam rings, each of which interact with acidic residues. c, Cutaway surface view of CXCR4 orthosteric pocket. The CXCL12 N terminus is shown in stick representation and AMD3100 is shown in sphere representation to illustrate their relative binding positions in the orthosteric pocket.
Amino acid substitutions at the distal N terminus of CXCL12 can convert the chemokine into an antagonist40, highlighting the importance of the N terminus for receptor activation. Our structure shows how the CXCL12 N terminus protrudes into the orthosteric pocket of CXCR4 and makes extensive contacts with the TM core (Fig. 2a). The distal CXCL12 N terminus is positioned overall deeper into the pocket compared to the antagonistic viral chemokine vMIP-II (ref. 20) (Extended Data Fig. 4e), consistent with their respective ligand functions. P2CXCL12 penetrates deepest into the orthosteric pocket, contacting the side chain of Y1163.32 (Ballesteros–Weinstein numbering41 in superscript). The side chain of K1CXCL12 projects upward from the TM core to the extracellular side of the receptor and is positioned to interact electrostatically with D972.63 and possibly D187ECL2. S4CXCL12 makes an apparent hydrogen bond interaction with E2887.39. L5CXCL12 packs onto a mainly hydrophobic surface composed of L411.35, Y451.39, W942.60 and A982.64. R8CXCL12 appears poised to make a charge–charge interaction with D2626.58, as predicted previously on the basis of charge-swap experiments28. Several of the CXCR4 residues mentioned above (W942.60, D972.63, Y1163.32, D187ECL2 and E2887.39) have been shown to be important for CXCL12–CXCR4 signaling28,42,43, underscoring the functional relevance of the interactions observed in our cryo-EM structure. We expand on the structural basis of CXCL12 activation of CXCR4 in a later section.
We observed unambiguous density for the bilobed AMD3100 molecule in our cryo-EM reconstruction of CXCR4EM–Gi–AMD3100 (Fig. 1c). Although it has primarily been described as an antagonist44, our observation that AMD3100 binds to the active CXCR4EM–Gi complex without disrupting G-protein coupling is consistent with the compound acting as a weak partial agonist on constitutively active mutants of CXCR4 (ref. 23). AMD3100 binds the orthosteric pocket using a diagonal orientation and directly blocks CXCL12 docking, although its overall binding mode is shifted toward TM5 and TM6 relative to the CXCL12 N terminus (Fig. 2b,c). Each of the two positively charged cyclam rings of AMD3100 (ref. 45) is stabilized electrostatically by an acidic side chain pointed toward the center of the ring; the cyclam moiety closer to the extracellular side interacts with D2626.58 while the cyclam proximal to the TM core interacts with E2887.39. The closely matched spacings between the side chains of D2626.58 and E2887.39 residues and the cyclam rings, therefore, appear be the main binding determinant of AMD3100 and other bicyclam analogs. Consistent with our structure, a previous study showed that that D262N and E288A mutants each reduced the affinity of AMD3100 to CXCR4 more than 50-fold45. The central aromatic ring of the phenylenebis(methylene) linker connecting the two cyclam moieties makes hydrophobic contacts with I2847.35, which is positioned directly in between D2626.58 and E2887.39 in the orthosteric pocket. This interaction may contribute to the increased potency of bicyclams with an aromatic linker relative to those with an aliphatic linker46. The rigidity imposed by the aromatic linker on the relative positions of the two cyclam moieties may also have a role in stabilizing the binding pose of AMD3100.
Antagonism of CXCR4 by REGN7663 monoclonal antibody
Antibody-based therapeutics against CXCR4 and other GPCRs are a promising alternative to small molecules because of their high specificity to the target, opportunity for Fc effector functions and favorable pharmacokinetic properties11,13,47,48. REGN7663 is a fully human anti-CXCR4 monoclonal antibody generated using VelocImmune mice49,50. We showed using a cyclic adenosine monophosphate response element (CRE) luciferase reporter assay that REGN7663 is a potent blocker (half-maximal inhibitory concentration (IC50) = 2.7 ± 0.1 nM, calculated from n = 3 independent experiments) of CXCL12-induced signaling in HEK293 cells engineered to overexpress CXCR4 (Fig. 3a). Furthermore, in the absence of CXCL12, REGN7663 decreased the apparent basal activity (half-maximal effective concentration (EC50) = 1.3 ± 0.4 nM, calculated from n = 3 independent experiments), indicating inverse agonism in the setting of CXCR4 overexpression (Fig. 3b). Notably, we observed that the dose–response curve for REGN7663 showed a Hill slope of approximately 1 in agonist mode (in the absence of CXCL12) and a Hill slope of approximately 2 in antagonist mode (in the presence of 0.5 nM CXCL12). Understanding the molecular basis for this apparent difference in cooperativity will require additional study.

a, CRE luciferase reporter assay showing CXCL12-dependent decrease in signal and block of CXCL12 activity (at 0.5 nM CXCL12) by REGN7663 (light blue). The negative control monoclonal antibody (violet) showed no effect. The IC50 for REGN7663 was calculated to be 2.7 ± 0.1 nM (mean ± s.d.) in antagonist mode from n = 3 independent experiments. RLU, relative luminescence units. b, REGN7663 shows a concentration-dependent increase in signal relative to baseline in the absence of CXCL12, demonstrating inverse agonism. The EC50 for REGN7663 was calculated to be 1.3 ± 0.4 nM in agonist mode (absence of CXCL12) from n = 3 independent experiments. In a,b, representative data from one experiment are shown (the same data for CXCL12 are shown as solid black circles in a,b to allow a comparison to monoclonal antibody data). c, Cryo-EM reconstruction of CXCR4EM–Gi–REGN7663 Fab complex, with each polypeptide chain colored differently. d, Top-down view of CXCR4 (yellow) with CDR loops of bound REGN7663 shown (blue, heavy chain (HC); cyan, light chain (LC)). e, Electrostatic interaction between CDR-H3 of REGN7663 and CXCR4 orthosteric pocket-facing residue E288.
Source data
To understand how REGN7663 binds and inhibits CXCR4, we determined a 3.4-Å-resolution cryo-EM structure of REGN7663 Fab in complex with CXCR4EM–Gi (Fig. 3c, Extended Data Fig. 5a,d, Table 1 and Supplementary Fig. 1). The structure revealed that REGN7663 binds directly onto the extracellular face of CXCR4, antagonizing the receptor by steric blockade of CXCL12 binding. Most of the REGN7663 epitope resides at the extracellular N-terminal region and ECL2 (Extended Data Fig. 5e,f). The REGN7663 heavy chain dominates the binding interactions, burying more surface area (~1,100 Å2) than the light chain (~300 Å2). Although the overall architecture of the complex is similar to the apo, CXCL12-bound and AMD3100-bound CXCR4EM–Gi structures, REGN7663 binding induces distinct conformations of the N terminus and ECL2, suggesting that their flexibility is important for specific monoclonal antibody binding (Extended Data Fig. 5g). Heavy-chain complementarity-determining regions (CDRs) 1 and 2 of REGN7663 are oriented toward the extracellular ends of TM4 and TM5, while light-chain CDRs are oriented extracellular to TM1 and TM2 (Fig. 3d). Remarkably, the CDR-H3 loop of REGN7663 wedges between the CXCR4 N terminus and ECL2, exhibiting a partial insertion into the CXCR4 orthosteric pocket. The side chain of REGN7663 residue R105 protrudes deepest into the orthosteric pocket, making an apparent charge–charge interaction with E2887.39(Fig. 3e). The insertion of the CDR-H3 loop, albeit not activating in the case of REGN7663, is reminiscent of how the CDR3 loop of the single-domain antagonist antibody JN241 occupies the orthosteric pocket of apelin receptor51. Taken together with the finding that JN241 was converted into a full agonist through subtle engineering of CDR3 (ref. 51), our structure of the REGN7663–CXCR4 complex illustrates the potential for full antibodies (containing light and heavy chains) functionally modulating GPCRs by inserting CDR loop(s) into the orthosteric pocket.
CXCR4 activation and Gαi protein docking
We next sought to assess the conformational changes associated with CXCR4 activation. Available crystal structures of inactive, antagonist-bound CXCR4 contain construct modifications (namely T4 lysozyme (T4L) inserted at intracellular loop 3 (ICL3) and a thermostabilizing amino acid substitution in TM3) that could confound comparison to our current structures. We, therefore, determined a 3.1-Å-resolution cryo-EM structure of CXCR4EM in the absence of Gi protein, using REGN7663 Fab as a fiducial mark (Fig. 4a, Extended Data Fig. 5h–k, Table 1 and Supplementary Fig. 2). Structural alignment of the REGN7663 Fab–CXCR4EM–Gi structure with the Gi-free REGN7663 Fab–CXCR4EM structure showed nearly identical conformations at the REGN7663 epitope and paratope regions but distinct conformations at the intracellular half of the receptor, including the characteristic movement of TM6 underlying receptor activation (Fig. 4b and Extended Data Fig. 5l). Additional conformational changes upon activation and Gi binding include the movement of TM5 toward TM6, subtle displacement of TM2 outward, an inward kink of TM7 and loss of ordered structure in H8. We note that H8 was also unresolved in previously determined antagonist-bound CXCR4 crystal structures19,20, suggesting that this is a consistent feature of the inactive receptor.
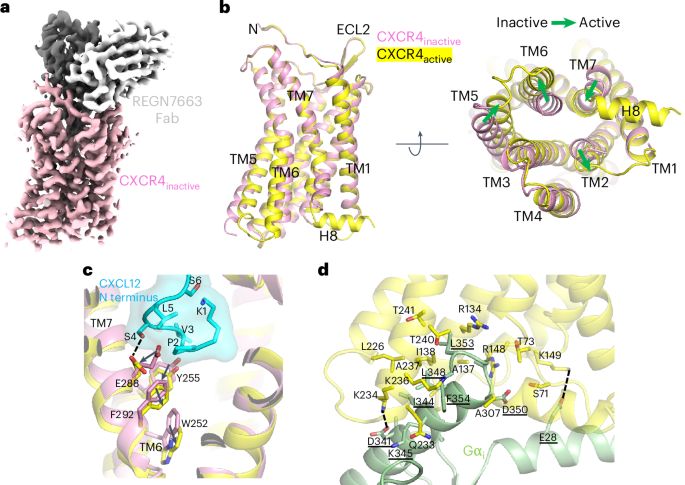
a, Cryo-EM reconstruction of inactive CXCR4EM–REGN7663 Fab complex (CXCR4, pink; REGN7663 heavy chain, gray; REGN7663 light chain, white). b, Structural alignment of inactive CXCR4 (pink) and active CXCR4 (yellow); the CXCR4EM–Gi–REGN7663 Fab complex was used for alignment. Left, side view; right, bottom-up view. The green block arrows depict conformational transitions from inactive to active CXCR4. c, Expanded view showing CXCL12 N terminus (cyan) binding to active CXCR4 (yellow). Inactive CXCR4 (pink) is shown for comparison and residues important for transmitting chemokine binding into activation are shown in stick representation. d, Expanded view of Gαi (light green) binding to active CXCR4 (yellow). Residues participating in the interaction are shown in stick representation and labeled (Gαi residue labels are underlined). Electrostatic interactions are highlighted with dashed lines.
We further compared the conformations of the inactive and CXCL12-bound structures to analyze how CXCL12 binding results in activation (Fig. 4c). Binding of the CXCL12 N-terminal coil to the orthosteric pocket requires structural changes to the inactive state pocket. Residues P2 and S4 at the CXCL12 N terminus push E2887.39 outward and toward the cytoplasmic side, while V3CXCL12 forces a downward displacement of Y2556.51. The movements of E2887.39 and Y2556.51 are in turn transmitted to F2927.43, which was previously implicated in CXCR4 signal transmission43, and conserved toggle switch residue52 W2526.48, respectively. Together, these conformational changes trigger further structural rearrangements that ultimately stabilize the active, Gi-bound conformation of CXCR4. Furthermore, a close comparison revealed that, because of binding of the CXCL12 N terminus in the orthosteric pocket, the E2887.39 side chain reorients, along with a small, ~0.7–1 Å outward movement of the extracellular half of the TM7 helix relative to our AMD3100–CXCR4EM–Gi, REGN7663 Fab–CXCR4EM–Gi and apo CXCR4EM–Gi structures (Extended Data Fig. 6a). This slight conformational difference at TM7 induced by CXCL12 may explain why it is a full agonist while the other ligands are not. Similar structural mechanisms of chemokine activation to that described above for CXCL12 were observed for the CCR2–CCL2 complex32 and CCR5–MIP-1α complex34.
Like other class A GPCRs, coupling of Gαi to CXCR4 is mediated by insertion of the Gαi α5 helix and C-terminal ‘wavy hook’ into the cytoplasmic-facing core of the receptor TM domain (Fig. 4d). Wavy hook residues L353 and F354 bury deepest into CXCR4 and contact R1343.50, Q233ICL3, K2366.32, A2376.33, T2406.36 and A307 mainly through van der Waals and hydrophobic interactions. The Gαi α5 helix makes numerous additional contacts with TM2, TM3, ICL2, TM5, ICL3 and TM6. Salt-bridge interactions involving D341(Gαi)–K2346.30 and E28(Gαi)–K1494.38 probably have an important role in stabilizing the docking of Gi protein onto CXCR4. Although the overall Gi binding mode of CXCR4 and other chemokine receptors is shared, the angle at which the Gαi α5 helix docks into the TM bundle differs slightly (Extended Data Fig. 6b). While CXCR4, CXCR1 (ref. 36) and CXCR2 (ref. 31) show highly similar α5 docking angles, the docking angles in CCR1 (ref. 33), CCR2 (ref. 32) and CCR5 (ref. 34) are similar to each other and shifted relative to CXCR4 because of distinct intracellular loop conformations and receptor interactions with Gαi (Extended Data Fig. 6c). More specifically, in the CC chemokine receptors, the Gαi α5 helix is shifted toward ICL2 and further from ICL3. Available data, therefore, indicate that CXC and CC chemokine receptors have slightly different Gi docking geometries.
Oligomeric structures of CXCR4
Although GPCRs are generally understood to function as monomeric units, numerous studies have reported that chemokine receptors form dimers and higher-order oligomers at the cell surface as expression levels increase53,54,55,56. Homo-oligomerization and hetero-oligomerization have been proposed to add complexity to chemokine receptor function, perhaps through allosteric communication between interacting subunits57,58. Multiple structures of CXCR4 from different crystal forms showed the same homodimeric architecture19,20, demonstrating that the detergent-solubilized receptor has the propensity to dimerize using specific intersubunit interactions mainly involving TM5 and TM6. Our SEC data of CXCR4EM consistently showed multiple peaks with different elution volumes, including peaks corresponding to oligomeric species larger than monomeric CXCR4EM or CXCR4EM–Gi (Extended Data Figs. 1b and 2a). Wild-type CXCR4 fused to GFP showed a similar FSEC profile to CXCR4EM, indicating that the apparent oligomerization was not specific to the constitutively active N119S substitution present in CXCR4EM.
We isolated and characterized a presumed oligomeric SEC peak (Extended Data Fig. 2a) of CXCR4EM using cryo-EM. Initial cryo-EM data yielded clear top and bottom views of trimeric and tetrameric species but the preferred orientation precluded structure determination. After screening various sample preparation conditions, we ultimately used stage-tilted data collection59 to obtain 3.4-Å-resolution reconstructions of CXCR4EM homotrimers and homotetramers in complex with REGN7663 Fab (Fig. 5, Extended Data Fig. 7a–j, Table 1 and Supplementary Fig. 2). According to three-dimensional (3D) classification, our data contained a roughly 1:3 ratio of trimers to tetramer particles (Extended Data Fig. 7k). We did not observe two-dimensional (2D) or 3D class averages consistent with dimeric CXCR4, except for nonphysiological antiparallel dimers in our samples prepared in the presence of Gi (Extended Data Fig. 7i). The trimer and tetramer both show CXCR4 subunits arranged symmetrically around a cavity at the central axis, at first glance evoking structural similarity to homomeric ion channels, although CXCR4 has no known channel function. In the case of the CXCR4 oligomers, we found evidence for numerous bound lipids at the central axis in the cryo-EM maps (Fig. 5c,f and Extended Data Fig. 8). Because of matching shape features, we tentatively built three phosphatidic acids and three cholesterol molecules in the trimeric map central cavity and four phosphatidic acids and eight cholesterols in the tetrameric cavity (Extended Data Fig. 8d,h). Although the presumed cholesterol molecules could, in principle, correspond to exogenously added cholesteryl hemisuccinate present in the purification buffers, the EM density we modeled as phosphatidic acid strongly resembles a phospholipid and not the LMNG detergent used for purification. This implies that the central cavity lipids were carried over from the cell membrane and remained stably bound through purification, indicating that the oligomeric structures reported here are representative of species present in the CXCR4-expressing cells used in this study and not an artifact of the purification process. The presence of ordered lipids plugging the central axis of CXCR4 oligomers is reminiscent of microbial channelrhodopsin trimers, although the quaternary arrangement of the seven-TM-helix protomers differs60,61.
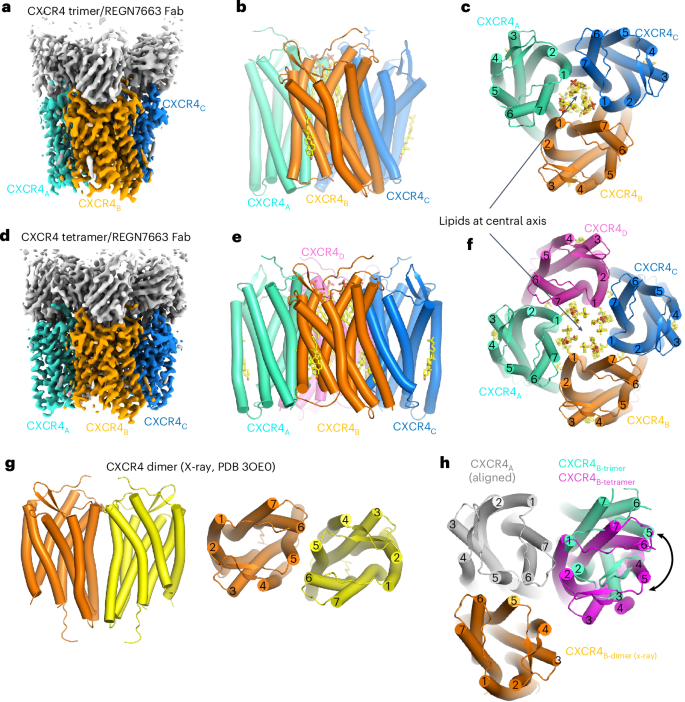
a, Cryo-EM reconstruction of CXCR4 trimer in complex with REGN7663 Fab. b,c, Side (b) and top-down (c) views of CXCR4 trimer structure. TM helices are shown in cylinder representation and bound lipids are shown in stick representation. Fab molecules are omitted for clarity. d, Cryo-EM reconstruction of CXCR4 tetramer in complex with REGN7663 Fab. e,f, Side (e) and top-down (f) views of CXCR4 tetramer structure. g, Side (left) and top (right) views of previously reported dimeric crystal structure of CXCR4. h, Top-down view of a CXCR4 protomer (gray) showing the positions of neighboring subunits from a dimer (orange), trimer (cyan) and tetramer (magenta).
The comparable interprotomer interfaces of trimeric and tetrameric CXCR4 are composed of TM5, TM6 and TM7 of one protomer interacting with TM1 and TM7 of the neighboring protomer (Fig. 5c,f). A ~20° rotation of the angle between neighboring subunits underlies the distinct oligomeric states (Fig. 5h). This oligomeric interface does not overlap with the dimeric interface observed in crystal structures of CXCR4 (refs. 19,20) (Fig. 5h), speculatively allowing for ‘superclustering’ of CXCR4 protomers mediated by a combination of trimeric or tetrameric and dimeric interfaces (Extended Data Fig. 9a,b). The structural superposition indicates that the steric clash caused by the T4L fusion in the crystallization construct may have precluded the assembly of trimers or tetramers observed in our data (Extended Data Fig. 9c,d), thus suggesting why homodimer formation was favored for the T4L-fused receptor.
The trimeric interface is characterized by a buried surface area of ~1,150 Å2 and is primarily mediated by crisscrossing of TM6 and TM1 of neighboring protomers near the midpoint of the membrane (Fig. 6a). The diagonal orientation of TM6 results in interprotomer contacts with the cytoplasmic half of TM7. TM1 of the neighboring protomer makes additional interprotomer contacts with cytoplasmic end of TM5 and the extracellular tip of TM7. As expected from interactions between TM helices, most of the residues involved are hydrophobic. As noted above, the tetramer interface is similar to the trimer interface (Fig. 6b). However, close inspection revealed a remarkable difference in the tetramer: a sterol-shaped density that we tentatively built as cholesterol present at the cytoplasmic half of the bilayer sandwiched between TM5 and TM6 of one protomer and TM1 and TM7 of its neighbor (Fig. 6b,c). To make space for sterol binding at the tetrameric interface, the intracellular portion of TM6 splays away from the interface and TM1 of the neighboring protomer rotates relative to their conformations in the trimeric interface (Fig. 6d). The TM1 rotation is concurrent with the rotation of the entire CXCR4 protomer, which in turn allows space for the additional subunit present in the tetrameric assembly (Fig. 5h). Our structures, therefore, imply that the absence or presence of lipid at the CXCR4 interprotomer interface may drive the assembly of trimers and tetramers, respectively. These findings provide a structural example supporting the idea that cholesterol regulates chemokine receptor oligomerization62.
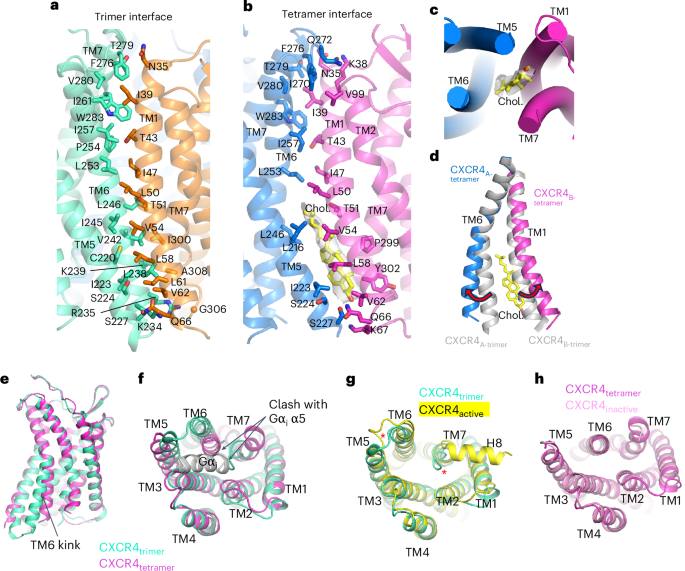
a, Interprotomer interface of CXCR4 trimer. Interface residues are shown in stick representation and labeled. b, Interprotomer interface of CXCR4 tetramer. Interface residues and modeled cholesterol are shown in stick representation. The density corresponding to cholesterol is shown as a transparent gray surface. c, Bottom-up view showing position of cholesterol at the tetramer interface. d, Structural alignment of TM6 and TM1 at the trimer (gray) and tetramer (blue and magenta, with cholesterol (yellow) shown in stick representation). e,f, Side (e) and bottom-up (f) views of protomeric structures of trimeric (cyan) and tetrameric (magenta) CXCR4. Binding of the Gαi α5 helix (gray) is prevented by steric clash. g, Structural alignment of trimeric CXCR4 protomer (cyan) and active CXCR4 protomer (yellow). Red asterisks highlight the distinct positions of ICL3 and TM7. h, Structural alignment of tetrameric CXCR4 protomer (magenta) and inactive CXCR4 (pink).
A super-resolution microscopy study reported that the simultaneous introduction of three substitutions (K239E, V242A and L246A) within TM6, located at the oligomerization interface observed in our structures, resulted in reduced higher-order oligomerization of CXCR4 (ref. 21). We used FSEC to examine the effect of this triple mutant and other substitutions at the oligomeric interface on the oligomerization behavior of the detergent-extracted receptor, using CXCR4EM as the background construct (Extended Data Fig. 10a). The K239E;V242A;L246A and K239E;V242W;L246W triple mutants both showed a reduced propensity to form oligomers relative to monomers, determined from the FSEC peak–area ratio of oligomer to monomer for each mutant (Extended Data Fig. 10b). We found that the single mutant V242W showed similarly reduced oligomerization, likely by introducing steric hindrance at the oligomerization interface. On the other hand, L246W increased apparent oligomerization and reduced monomer levels, possibly by augmenting the hydrophobic interactions between subunits. A substitution at a TM1 residue (L58W) that faces TM5 of the neighboring subunit also showed a reduced oligomer-to-monomer ratio. Other TM1, TM6 and TM7 mutants showed no notable change in oligomer-to-monomer ratio (T51W) or did not have clearly interpretable FSEC chromatograms, presumably because of impacts on expression level or stability of the receptor in detergent. Overall, these biochemical data corroborate the oligomeric interface observed in our structural data.
We next examined the conformations of the individual protomers within the CXCR4 trimer and tetramer. As noted above, a striking difference is the kink at TM6 associated with sterol binding (Fig. 6e,f). TM6 of the trimeric protomer is kinked outward relative to that of the tetrameric protomer, suggesting a more active-like conformation. Indeed, the structure of the trimeric protomer matches closely with active CXCR4 in complex with REGN7663 Fab and Gi, while the tetrameric protomer aligns well with the inactive CXCR4–REGN7663 Fab complex in the absence of Gi (Fig. 6g,h). A noteworthy distinction between the trimeric CXCR4 protomer and active, Gi-bound CXCR4 is the conformation of ICL3, TM7 and H8; in the trimer, ICL3 is pushed away from the cytoplasmic-facing core, the C-terminal end of TM7 is tucked inward, effectively blocking Gi binding, and H8 is not visible in the cryo-EM map (Fig. 6f,g). Therefore, while trimeric CXCR4 is composed of protomers with an active-like conformation, they are not structurally competent for Gi coupling and, as such, cannot be deemed fully active. This structural observation agrees with FSEC data showing that the presence of Gi did not result in a shift of the oligomeric peak (Extended Data Fig. 1b). Overall, these oligomeric structures demonstrate that distinct protomeric conformations underpin the trimeric and tetrameric arrangements of CXCR4. Lipids found at the central axis and at the tetrameric interface appear to be important for oligomeric assembly.